12
This unit will focus on how light (and in some cases electrons) travel through both empty space and matter as well as how those interactions can be used to manipulate the paths and make images. The most familiar optical system to most of you is the one you are probably using to read these very words: your eyes! We will therefore be looking at the eye quite a bit throughout this unit, both human eyes and simpler eyes in the animal kingdom. Another common biological application of optics is the microscope. To make sure that everyone is on the same page, you will find below some information about the anatomy of the human eye as well as some basic information about microscopes. Please be familiar with this terminology as we will use it in class.
The Human Eye. Derived from 36.5 Vision by OpenStax Biology
Vision is the ability to detect light patterns from the outside environment and interpret them into images. Animals are bombarded with sensory information, and the sheer volume of visual information can be problematic. Fortunately, the visual systems of species have evolved to attend to the most-important stimuli. The importance of vision to humans is further substantiated by the fact that about one-third of the human cerebral cortex is dedicated to analyzing and perceiving visual information.
Anatomy of the Eye
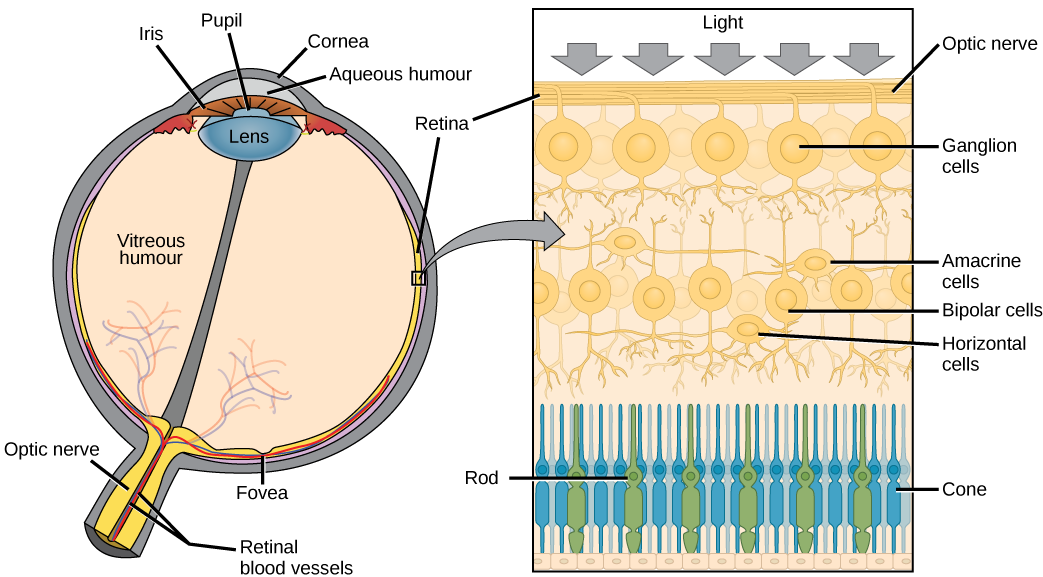
Changes in material are crucial to image formation using lenses. Each material has an index of refraction which will be discussed in The Ray Aspect of Light: The Speed of Light in Materials. The biggest change in material, and consequently the biggest bending of rays, actually occurs at the cornea rather than the lens. The cornea provides about two-thirds of the power of the eye, owing to the fact that speed of light changes considerably while traveling from air into cornea. The lens provides the remaining power needed to produce an image on the retina. The cornea and lens can be treated as a single thin lens, even though the light rays pass through several layers of material (such as cornea, aqueous humor, several layers in the lens, and vitreous humor), changing direction at each interface. The image formed is much like the one produced by a single convex lens.
The goal of the cornea and lens is to focus light on the retina and, in particular, the fovea centralis. The fovea centralis is a small, central pit composed of closelpacked cones in the eye. The fovea is responsible for sharp central vision, which is necessary in humans for activities for which visual detail is of primary importance, such as reading and driving. The lens is dynamic, focusing and re-focusing light as the eye rests on near and far objects in the visual field. The lens is operated by muscles that stretch it flat or allow it to thicken, changing the focal length of light coming through it to focus it sharply on the retina. With age comes the loss of the flexibility of the lens, and a form of farsightedness called presbyopia results. Presbyopia occurs because the image focuses behind the retina. Presbyopia is a deficit similar to a different type of farsightedness called hyperopia caused by an eyeball that is too short. For both defects, images in the distance are clear but images nearby are blurry. Myopia (nearsightedness) occurs when an eyeball is elongated and the image focus falls in front of the retina. In this case, images in the distance are blurry but images nearby are clear.
There are two types of photoreceptors in the retina: rods and cones, named for their general appearance as illustrated in . Rods are strongly photosensitive and are located in the outer edges of the retina. They detect dim light and are used primarily for peripheral and nighttime vision. Cones are weakly photosensitive and are located near the center of the retina. They respond to bright light, and their primary role is in daytime, color vision.
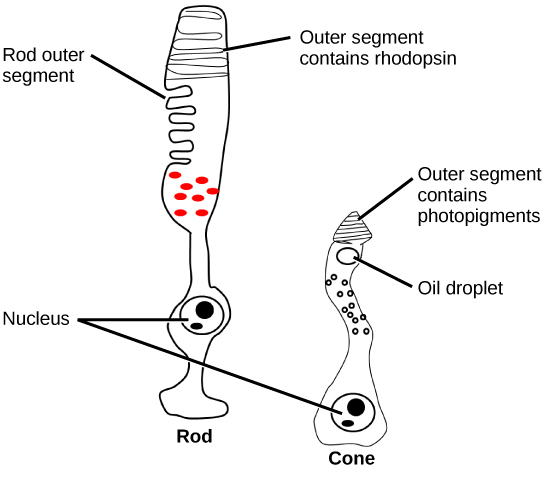
The fovea is the region in the center back of the eye that is responsible for acute vision. The fovea has a high density of cones. When you bring your gaze to an object to examine it intently in bright light, the eyes orient so that the object’s image falls on the fovea. However, when looking at a star in the night sky or other object in dim light, the object can be better viewed by the peripheral vision because it is the rods at the edges of the retina, rather than the cones at the center, that operate better in low light. In humans, cones far outnumber rods in the fovea.
Problem 1: Identify the portion of the eye responsible for most of the focusing of light.
Problem 2: What is the purpose of the iris?
Transduction of Light
The rods and cones are the site of transduction of light to a neural signal. Both rods and cones contain photopigments. In vertebrates, the main photopigment, rhodopsin, has two main parts (Figure 4) an opsin, which is a membrane protein (in the form of a cluster of α-helices that span the membrane), and retinal—a molecule that absorbs light. When light hits a photoreceptor, it causes a shape change in the retinal, altering its structure from a bent (cis) form of the molecule to its linear (trans) isomer. This isomerization of retinal activates the rhodopsin, starting a cascade of events that ends with the closing of Na+ channels in the membrane of the photoreceptor. Thus, unlike most other sensory neurons (which become depolarized by exposure to a stimulus) visual receptors become hyperpolarized and thus driven away from threshold (Figure 5).
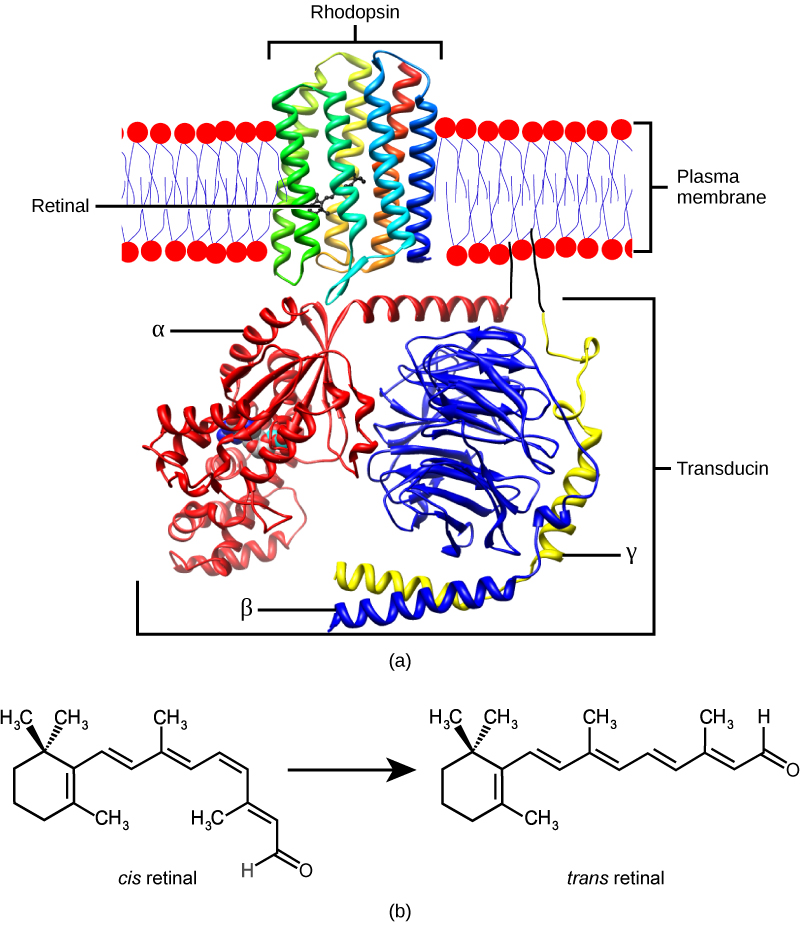
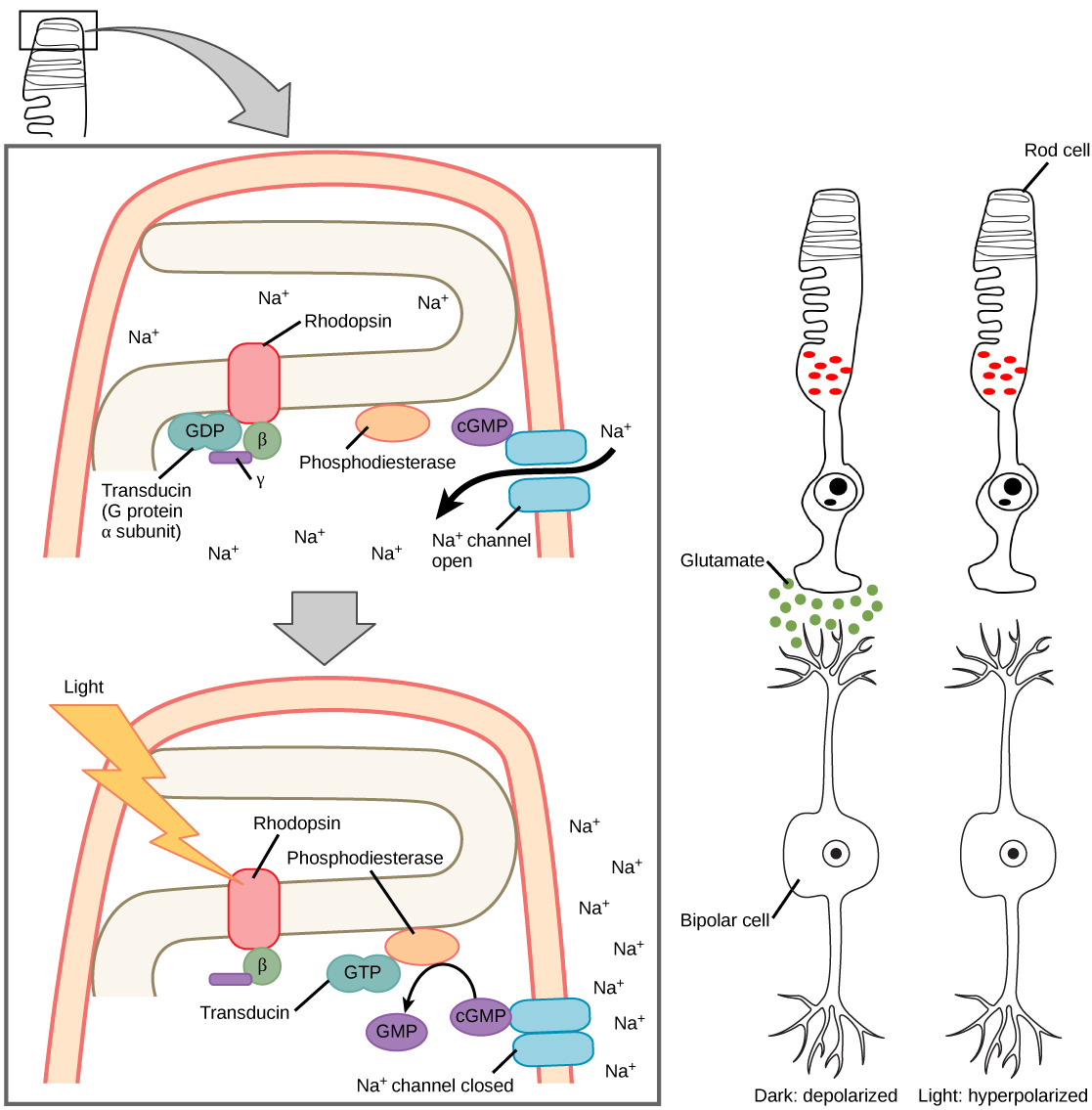
Trichromatic Coding
There are three types of cones (with different photopsins), and they differ in the wavelength to which they are most responsive, as shown in Figure 6 . Some cones are maximally responsive to short light waves of 420 nm, so they are called S cones (“S” for “short”); others respond maximally to waves of 530 nm (M cones, for “medium”); a third group responds maximally to light of longer wavelengths, at 560 nm (L, or “long” cones). With only one type of cone, color vision would not be possible, and a two-cone (dichromatic) system has limitations. Primates use a three-cone (trichromatic) system, resulting in full color vision. The color we perceive is a result of the ratio of activity of our three types of cones. The colors of the visual spectrum, running from long-wavelength light to short, are red (700 nm), orange (600 nm), yellow (565 nm), green (497 nm), blue (470 nm), indigo (450 nm), and violet (425 nm). Humans have very sensitive perception of color and can distinguish about 500 levels of brightness, 200 different hues, and 20 steps of saturation, or about 2 million distinct colors.
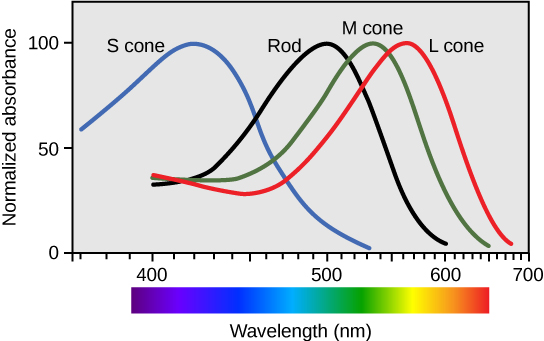
Retinal Processing
Visual signals leave the cones and rods, travel to the bipolar cells, and then to ganglion cells. A large degree of processing of visual information occurs in the retina itself, before visual information is sent to the brain.
Photoreceptors in the retina continuously undergo tonic activity. That is, they are always slightly active even when not stimulated by light. In neurons that exhibit tonic activity, the absence of stimuli maintains a firing rate at a baseline; while some stimuli increase firing rate from the baseline, and other stimuli decrease firing rate. In the absence of light, the bipolar neurons that connect rods and cones to ganglion cells are continuously and actively inhibited by the rods and cones. Exposure of the retina to light hyperpolarizes the rods and cones and removes their inhibition of bipolar cells. The now active bipolar cells in turn stimulate the ganglion cells, which send action potentials along their axons (which leave the eye as the optic nerve). Thus, the visual system relies on change in retinal activity, rather than the absence or presence of activity, to encode visual signals for the brain. Sometimes horizontal cells carry signals from one rod or cone to other photoreceptors and to several bipolar cells. When a rod or cone stimulates a horizontal cell, the horizontal cell inhibits more distant photoreceptors and bipolar cells, creating lateral inhibition. This inhibition sharpens edges and enhances contrast in the images by making regions receiving light appear lighter and dark surroundings appear darker. Amacrine cells can distribute information from one bipolar cell to many ganglion cells.
You can demonstrate this using an easy demonstration to “trick” your retina and brain about the colors you are observing in your visual field. Look fixedly at Figure 7 for about 45 seconds. Then quickly shift your gaze to a sheet of blank white paper or a white wall. You should see an afterimage of the Norwegian flag in its correct colors. At this point, close your eyes for a moment, then reopen them, looking again at the white paper or wall; the afterimage of the flag should continue to appear as red, white, and blue. What causes this? According to an explanation called opponent process theory, as you gazed fixedly at the green, black, and yellow flag, your retinal ganglion cells that respond positively to green, black, and yellow increased their firing dramatically. When you shifted your gaze to the neutral white ground, these ganglion cells abruptly decreased their activity and the brain interpreted this abrupt downshift as if the ganglion cells were responding now to their “opponent” colors: red, white, and blue, respectively, in the visual field. Once the ganglion cells return to their baseline activity state, the false perception of color will disappear.
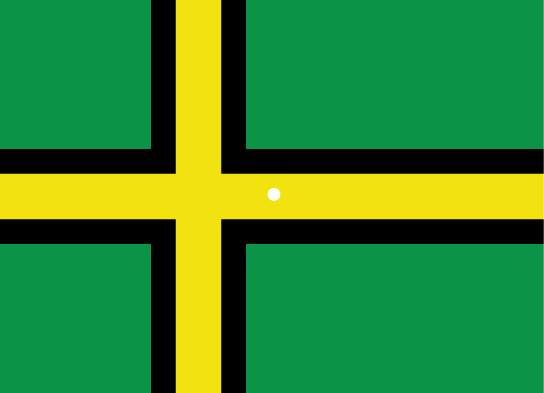
The apparent reproduction of an object, formed by an optical element (or collection of them) reflecting and/or refracting light.
sense of sight
layer of photoreceptive and supporting cells on the inner surface of the back of the eye
The transparent layer over the front of the eye that helps focus light waves. Most of the focusing of the eye actually happens at the cornea, not in the lens.
The transparent, convex structure behind the cornea that helps focus light waves on the retina. The lens is for the fine-tuning.
The pigmented, circular muscle at the front of the eye that regulates the amount of light entering the eye.
The small opening at the front of the eye though which light enters. Appears black (or red in flash photographs!). The size is controlled by the iris.
Visual defect in which the image focus falls behind the retina, thereby making images in the distance clear, but close-up images blurry; caused by age-based changes in the lens.
Visual defect in which the image focus falls behind the retina, thereby making images in the distance clear, but close-up images blurry.
Visual defect in which the image focus falls in front of the retina, thereby making images in the distance blurry, but close-up images clear
Strongly photosensitive, achromatic, cylindrical neuron in the outer edges of the retina that detects dim light and is used in peripheral and nighttime vision. Can only see in black and white.
A set of weakly photosensitive, cone-shaped neurons in the fovea of the retina that detects bright light and is used in daytime color vision. There are cones responsible for red light, green light, and blue light.
region in the center of the retina with a high density of photoreceptors and which is responsible for acute vision
main photopigment in vertebrates
in a neuron, slight continuous activity while at rest